Magnetic resonance
spectroscopy of the neonatal
brain
Nicola J Robertson and I Jane Cox
Chapter Contents
- Technical aspects
- Normal brain development in infants and children
- Cerebral pathology
- Conclusions
- Summary
- References
Technical aspects
INTRODUCTION
Nuclear magnetic resonance (NMR) spectroscopy is one of the most important tools for quantitative analysis of chemical composition and structure; in the last two decades this technique has been applied in vivo to study biochemical processes in the preterm and term infant brain. Interpretation of a clinical MR spectrum can provide information about cellular energetics, membrane turnover, neuronal function, selected neurotransmitter activity, the fate of anesthetic agents and of certain drugs.
The first set of 1.5–2.0 Tesla (T) horizontal magnets in the early 1980s had a bore of approximately 20cm, so it was feasible to study cerebral metabolism only in newborn infants15. It is now possible to obtain clinical MR spectra using whole-body 1.5–2.0 T MR systems 24, either as an adjunct to an MRI examination or as a separate study. The availability of routine clinical MRI systems with localized spectroscopy capabilities has considerably widened the applicability of clinical magnetic resonance spectroscopy (MRS). On some systems, generally those in a research setting, there is scope for obtaining spectra from a number of different nuclei, which allow different aspects of in vivo biochemistry to be studied.
OBTAINING MULTINUCLEAR SPECTRA
The NMR phenomenon underlies both MRI and clinical MRS34. While MRI demonstrates anatomy and highlights structural abnormalities, MRS may be used to obtain non-invasive metabolic information in health and disease. Certain atomic nuclei, such as hydrogen-1 (proton, 1H), phosphorus-31 (31P), carbon-13 (13C), fluorine-19 (19F) and nitrogen-15 (15N), can be induced to produce radiofrequency signals in the presence of a strong magnetic field. In a static magnetic field (B0) these nuclei become weakly magnetized along the direction of the field and can be imagined to be acting like tiny bar magnets. An MR signal is generated by applying a second time-dependent magnetic field (B1), which changes the direction of magnetization. The induced magnetization then relaxes back to the original direction after the B1 pulse has been removed, producing the MR signal which is known as the free induction decay (FID). The resonance frequency of a nucleus is primarily influenced by its magnetogyric ratio as well as B0. For MRS applications, the FID can be resolved into a frequency spectrum by the mathematical function of Fourier transformation. The local magnetic environment of a nucleus and therefore the resonance frequency is subtly influenced by its immediate chemical environment. The relative frequency position can be described by a parameter known as chemical shift, a dimensionless unit accounting for the strength of the static magnetic field and measured in parts per million (ppm). In principle, the intensity of the metabolite signal is directly related to its concentration, but in practice absolute quantitation is difficult to achieve in vivo14. Many in vivo MRS studies, therefore, report results in terms of metabolite ratios. Since the technique is relatively insensitive only compounds present in millimolar concentrations are detectable using clinical in vivo MRS.
The phosphorus nucleus has proved to be particularly valuable in clinical in vivo MRS since it is technically straightforward to collect a spectrum and because the resonances observable are from phosphate groups in metabolites of central importance in energy metabolism.
The proton is the most common nucleus in biological systems and has the highest absolute NMR sensitivity. In vivo 1H MRS studies are a technical challenge for a number of reasons: (i) the water signal is 10000 times larger than signals from metabolites of interest; (ii) the chemical shift encountered covers a narrow range, so peak overlap is a problem. Furthermore there are stringent demands on magnetic field homogeneity; interaction between nearby protons within a molecule (spin–spin coupling) complicates the spectral pattern; and (iii) the scalp lipid signal is 100 times larger than the metabolite signals and produces broad features which can overlap and partially obscure the sharper resonances from smaller, more mobile species.
HARDWARE REQUIREMENTS
A clinical MR system comprises a magnet, shim coils, gradient coils, a radiofrequency transmitter/receiver system and a computer to perform the function of equipment control, acquisition, processing and data storage. For MRS applications, a multinuclear radiofrequency capability is required if nuclei other than protons are to be studied.
The static magnetic field needs to be at least 1 T to achieve adequate sensitivity within a clinical examination time, and also to achieve adequate dispersion of signals from different moieties.
A critical step prior to the acquisition of spectroscopy data is the process known as shimming, by which the magnetic field is made as uniform as possible within the selected volume of interest.
SEQUENCES
An important prerequisite for the detailed interpretation of a clinical MR spectrum is some knowledge of the region from which the spectrum is obtained. Combining an MRS study with an MRI examination allows definition and visualization of the region of interest. Spatial localization in MRS has generally been achieved by one or more of the following four techniques: image selected in vivo spectroscopy (ISIS), point-resolved spectroscopy (PRESS), stimulated echo acquisition mode (STEAM) and phase encoding (also known as chemical shift imaging, [CSI]). The first three are generally single volume techniques: the ISIS method is often used for 31P MRS and the STEAM and PRESS methods are used for 1H MRS in conjunction with water suppression techniques. Spectroscopic information can be obtained simultaneously from a number of voxels in the whole slice or volume using CSI methods.
As mentioned previously, the appearance of a spectrum is influenced by interactions between neighboring nuclei, which causes line splitting of the NMR signal. This interaction, known as spin–spin coupling, is generally stronger the closer the nuclei. Some nuclei are adjacent to nuclei with a zero magnetic moment and therefore the resonance is a single peak, for example the N-(CH3)3 peak in choline-containing compounds. The coupling pattern however can become very complex as is the case for the –CH2-protons in glutamate and glutamine. If there is a time delay between excitation and the start of sampling of the FID (time to echo (TE)), for example if localization sequences are required, the phase of the coupled peaks is modulated and the resultant pattern will depend on both the delay before data acquisition and the coupling pattern. A good example of this is lactate; the methyl group in lactate is a doublet which will be inverted when the delay before data acquisition is 135 ms and upright when the delay is 270ms.
Various sequences have been developed for minimizing the water signal in proton MRS, for example using chemical shift excitation pulses (CHESS) preceding the localization pulses, the excited transverse magnetization of the water is nulled by dephasing with spoiler gradients prior to the spatial localization sequence. Another approach is to use frequency selective excitation pulses, which have a null in the region of the water resonance and maximum excitation in the region of the metabolite signals, for example the 1331 binomial pulses. The water signal has a shorter T2 than the signal from metabolites so using a T2 weighted sequence will additionally suppress the water signal.
Suppression of scalp lipid signals may become necessary when the volume of interest is near to the brain surface, or when phase encoding techniques are used and voxels containing lipid are included in the matrix. The lipid signal can be separated from the lactate doublet by exploiting differences in relaxation properties and phase modulation characteristics; a long delay before data acquisition will preferentially reduce signals from lipids.
QUANTITATION
The NMR measurement of absolute in vivo metabolite concentrations will provide a more detailed insight into basic neurochemistry and mechanisms of damage than can be achieved using measurement of peak area ratios. However, in order for the measurement of metabolite concentrations to be more useful than peak area ratios a longer examination time is needed to collect all the required data. This is particularly a problem for neonatal studies when the study time is dictated by the length of time the neonate will remain sufficiently still. Therefore a decision often has to be made between the range of data collected (e.g. MRI, 1H MRS and 31P MRS) and the extent to which specific spectral peak areas can be interpreted as metabolite concentrations.
Many MRS quantification techniques have been proposed and applied during the last 10 years10, 21, 27, 28, 30, 31, 50, 53, 54, 66, 82, 100. In general, a reference MR signal is required from a standard of known or assumed concentration and a knowledge of the relaxation parameters of the metabolites of interest are required to interpret the MR parameters from a specific spectrum as metabolite concentrations. A number of studies have been undertaken reporting the T1 and T2 values in neonates17, 18, 53, 100.
The routine applicability of three reference methods has been compared in a recent multicenter trial50. The three methods used were: (i) an internal water standard21, (ii) a phantom replacement method12,30; and (iii) a combined external standard/compartmental analysis method31, 54. Using the internal standard the reference signal was collected from water, one of the compounds naturally existing in the region of interest. Using the phantom replacement method, the position of the volume of interest was constant, the patient being replaced by an external standard. Using the external phantom method, a sample was placed next to the patient in the receiver coil, so the reference signal was obtained during the patient examination but using different regions of interest. Variations arising from differing proportions of cerebral spinal fluid (CSF), gray and white matter could be accounted for by using compartmental analysis. Only the internal water standard technique could be readily implemented at all participating sites and provided acceptable precision and interlaboratory reproducibility50.
The advantage of the internal water standard technique is that it is not necessary to compensate for any effects related to coil loading and to inhomogeneities in the pulse profile. There are no requirements for a phantom to be positioned, which can be practically difficult when the patient size varies considerably (e.g. comparing a preterm infant with a 1-month old healthy neonate). However, the internal water standard method requires knowledge of the MR-visible water concentration, that is not only what the water concentration is but how MR visible it is. For example, water bound to myelin is generally considered invisible since the T2 is 10–20ms61. Corrections are required for the proportion of CSF, gray and white matter within the region of interest and also the NMR visible water content of gray and white matter fractions. In practice, fully relaxed in vivo water signals need to be acquired from the same region of interest as the metabolite signals at a range of TE values. If it is assumed that the water signal consists of contributions from CSF (with a long T2) and water within the brain (with a short T2), then a biexponential fit to the full set of water echoes will allow calculation of the brain tissue water signal and the CSF water signal. A consideration for applying this method to clinical studies of the neonate is that both the brain water concentration and the MR visible fraction may change with age, particularly prematurity29, and also with pathology.
COMPARTMENTATION
Several types of cells are anatomically, biochemically and physiologically integrated to make up normally functioning brain tissue. The neuron is the communicating cell whose function is strongly shaped and sustained by the neuroglial cells. In a typical neuron, three major parts can be defined: i) the cell body or perikaryon which contains the nucleus and major cytoplasmic organelles ii) a variable number of dendrites which emanate from the perikaryon and ramify over a certain volume of gray matter and iii) a single axon which extends further from the perikaryon than the dendritic arbor. During development axons become surrounded by a myelin sheath which facilitates rapid impulse conduction. In addition to the ependyma lining the ventricles and central canal, there are three types of neuroglia or supporting cells in the brain: oligodendrocytes, astrocytes and microglia.
Cellular localization of function is referred to as compartmentation which may be either intercellular or intracellular. Due to the intrinsically low sensitivity of MR methods it is generally not possible in vivo to obtain spectra of single cells. Instead the spectrum is the sum of the signal from millions of cells and multiple cell types. In a few situations metabolic pathways and metabolites are localized to specific cell types and this can be seen using MRS. An example of this is the glutamate/glutamine neurotransmitter cycle in which glutamate released by neurons during neurotransmission is transported out of the synaptic cleft by surrounding glial cell membranes and converted to glutamine. This cycle is critical for normal brain function and protection against excitotoxicity. MRS measurements of isotopic labeling of metabolites involved allows information to be obtained about neuronal and glial metabolism and their interplay. This is discussed further in the section on glutamine and glutamate.
< prev | top | contents | next >
Normal brain development in infants and children

Fig. 16.1 31P MR spectra of (a) a normal preterm infant (GA 29+3 weeks (age at scan 35+1 weeks)), (b) a normal term infant, (c) an infant aged 6 months with normal neurodevelopmental outcome and (d) an adult control. These spectra illustrate the relative decrease in PME/ATP and increase in PDE/ATP with maturation.
Major maturational processes such as neuronal organization, proliferation, differentiation of glial cells and myelination occur before and after birth. These processes are reflected by changes occurring in brain metabolites detected by 31P and 1H MRS. Metabolite concentrations also vary in different parts of the brain, for example the white matter and basal ganglia. An understanding of age-dependent and regional changes in metabolite ratios and absolute concentrations of 31P and 1H metabolites is important to enable accurate identification and recognition of metabolite abnormalities in pathological states.
In the following section, peaks detected by in vivo 31P and 1H MRS are assigned followed by a discussion of the role of the individual metabolites.
PHOSPHORUS-31 (31P) MRS
The first 31P MR spectrum was obtained in 1982 in a preterm infant15. 31P MR spectra are generally taken from the whole brain because of the relatively low concentration.
Seven main peaks can be assigned in a cerebral phosphorus spectrum: phosphomonoesters (PME), inorganic phosphate (Pi), phosphocreatine (PCr), phosphodiesters (PDE) and the three phosphate groups (a, b, g) in nucleotide triphosphate (NTP) (Fig. 16.1). Notably absent from the spectrum are signals from membrane phospholipids such as phosphatidylcholine. The phosphorus nuclei in such large molecules have reduced mobility and the signals are NMR invisible. Similarly the signal from adenosine diphosphate (ADP) bound to proteins is not detectable using NMR methods, and therefore the small ADP signal observed only represents free ADP. The dominant contribution to the NTP peaks is from adenosine triphosphate (ATP), but 10–20% of the peak area may be due to guanosine triphosphate (GTP) and uridine triphosphate (UTP). An unresolved peak from nicotinamide adenine dinucleotide (NAD+/NADH) may be observed as a right-hand shoulder on the aATP resonance36. In clinical studies this shoulder is often difficult to distinguish from aATP.
31P MRS can also measure intracellular pH (pHi), a measurement which is difficult to obtain by other means in vivo.
Phosphomonoesters (PME) and phosphodiesters (PDE)
The peak assigned to PME is multicomponent, and may contain contributions from phosphoethanolamine (PE) and phosphocholine (PC), metabolites on the pathway of membrane synthesis, as well as intermediates of carbohydrate metabolism such as glucose-6-phosphate. The PDE signal predominantly contains contributions from glycerophosphoethanolamine (GPE) and glycerophosphocholine (GPC), which represent phospholipid breakdown products.
Spectra from a newborn infant are characterized by a high peak from PME relative to the lower concentrations of PCr, Pi and PDE (Fig. 16.1). PME decreases and PDE increases during the first 2–3 years after birth8, 103. The high PME peak at birth is attributed to the abundance of compounds destined for the production of membrane phospholipids and myelin whereas the PDE peak mainly represents phospholipid breakdown products. The change in the ratio of PME/PDE with maturation is therefore related to the process of myelination and proliferation and growth of glial cells. Quantification studies confirm this decrease in PME and increase in PDE with maturation; in addition there is a change in the chemical shift and width of the PME peak, indicating that the relative proportions of contributing metabolites, PE and PC, alter13 (Table 16.1).
Table 16.1 Concentrations of 31P metabolites in healthy brains of human neonates, infants and adults. (Reproduced with permission of the International Pediatric Research Foundation, Inc. from Buchli et al.13
Mean concentration ±SD (mmol/l = mmol/dm3) | |||||||
n | PME | Pi | PDE | PCr | ATP | 31P total | |
Neonates | 16 | 4.5 ± 0.7* | 0.6 ± 0.1† | 3.2 ± 0.8† | 1.4 ± 0.2† | 1.6 ± 0.2† | 14.9 ± 2.3† |
Infants | 17 | 3.6 ± 0.9 | 0.6 ± 0.1 | 4.2 ± 0.7 | 1.7 ± 0.3 | 1.8 ± 0.3 | 16.1 ± 2.5 |
Adults | 28 | 3.5 ± 0.6* | 1.0 ± 0.2† | 11.7 ± 2.2† | 3.4 ± 0.5† | 2.9 ± 0.4† | 29.3 ± 3.1† |
*Significant difference p <0.01 | |||||||
†Significant difference p <0.001 |
Phosphocreatine/inorganic phosphate (PCr/Pi)
When ATP generation is impaired, energy flux is maintained by the creatine kinase equilibrium with the breakdown of PCr and increase in Pi, so that a decline in the ratio of PCr/Pi is a valuable indicator of impaired energy metabolism even in the presence of normal or near normal ATP. PCr/Pi therefore gives a non-invasive measure of the adequacy of cerebral oxidative metabolism.
There is a significant increase with postnatal age in PCr/Pi in infants from 26 to 42 postconceptional weeks.
As seen from the metabolite concentrations in Table 16.1, this reflects a more pronounced increase in PCr compared with Pi13.
Adenosine triphosphate (ATP)
ATP is the energy currency of the cell and is produced more efficiently by oxidative phosphorylation (36 moles of ATP per mole of glucose) than anaerobic glycolysis (2 moles of ATP per mole of glucose). ATP concentrations have been presumed to be constant in the brain during maturation and have in the past been used as a stable reference to compare other metabolite changes103. This presumption was based on observations made in the biochemical analysis of the developing rat brain in which the cerebral [ATP] in both neonatal and adult rats were independent of age62, 67. However, caution may be necessary since in one in vivo study using a calibration phantom as a concentration standard, [ATP] was almost double in adult brain compared to a healthy neonate13.
Intracellular pH (pHi)
In 1973, Moon and Richards demonstrated that 31P MRS could be used to measure pHi – a key variable controlling cell metabolism and function69. The theory underlying this is that Pi is an acid which titrates in the physiological pH range. The acidic form of Pi resonates at 3.29ppm and the basic form at 5.68ppm; however, because the protonation – deprotonation reaction is extremely fast, usually a single peak is seen, the position of which depends on the relative concentrations of acid or base. The pHi is calculated from the chemical shift difference between Pi relative to PCr using the Henderson–Hasselbalch equation and a suitable titration curve, for example:
pHi = 6.77 + log | (Pi shift – 3.29) |
(5.68 – Pi shift) | |
where Pi shift = chemical shift difference between PCr and Pi (Fig. 16.2)81

Fig. 16.2 An expanded portion of a 31P MR spectrum demonstrating the chemical shift difference between Pi and PCr which is the basis of pHi measurement. The unit of measure of Pi shift is ppm (parts per million); ppm = 106 x (sample Hz – ref Hz)/ref Hz. The Pi chemical shift is independent of magnetic field strength allowing comparison of data from different spectrometers.
Estimation of pHi is accurate to about 0.1 pH units, and it is generally accepted that changes in pH can be measured to within 0.5 pH units or better34. Factors likely to affect the titration curve include temperature, ionic strength, presence of protein and the concentrations of sodium, potassium, phosphate, calcium and especially magnesium81.
The chemical shift of the PME peak is also pH-sensitive and can be used to measure the intracellular pHi in vivo, although at physiological pHi values (from pHi range 6.9 to 7.2) this is less sensitive than the Pi shift23.
The normal adult pHi has been reported to be 7.02 (+/–0.07) and this is thought to reflect the intracellular cytoplasmic pH. In our studies, normal infants born at term had a pHi of 7.05 +/– 0.06; in other studies however, the pHi was more alkaline at birth (7.11 +/– 0.06) with a subsequent decrease during postnatal maturation (Table 16.2)3, 13, 71.
At high field strengths and under certain physiological conditions such as hypothermia, the Pi peak can be resolved into extra- and intracellular83 and perhaps also mitochondrial35, 39 compartments, implying the presence of compartments of different pH. Our group has recently visualized a doublet Pi peak in some infants although it is presently unclear whether this doublet reflects extracellular and intracellular compartments83, neuronal/glial compartmentation98 or different intracellular compartments35.
Regional variation
Owing to the low sensitivity of the phosphorus nucleus and the large voxel size required, most neonatal 31P spectra are taken from the entire brain and cannot differentiate between gray and white matter. Regional differences in gray and white matter have been demonstrated in the adult and are likely to apply to the infant. Gray matter was found to have significantly higher ratios of PCr to g-ATP whereas the PCr/Pi ratio and pHi were not significantly different64.
Table 16.2 pHi in healthy brains of human neonates, infants and adults. (Reproduced with permission of the International Pediatric Research Foundation, Inc. from Buchli et al.13
n | Chemical shift difference shift (Pi-PCr) ppm | pHi* | |||||
---|---|---|---|---|---|---|---|
Neonates | 16 | 4.93 ± 0.07 | 7.11 ± 0.06† | ||||
Infants | 17 | 4.9 ± 0.08 | 7.09 ± 0.07 | ||||
Adults | 28 | 4.82 ± 0.08 | 7.02 ± 0.07† | ||||
| |||||||
†Significant difference: p <0.01. |
< prev | top | contents | next >
PROTON (1H) MRS
The first 1H MR spectrum from the neonatal brain was obtained in the early 1990s – almost a decade after the first neonatal 31P MR spectrum. The information obtained in a 1H MR spectrum is complementary to that obtained in a 31P MR spectrum. Because of the greater sensitivity of the 1H nucleus, data can be obtained from smaller regions of the brain. In addition, different TEs can be used to obtain more metabolite information. At short TEs, myo-inositol, Glx, lipids and macromolecules are more easily seen. Long TEs are usually used to obtain information about lactate as there will be less contamination from lipids and macromolecules.
Three singlet peaks can be readily assigned in the proton spectrum to N-acetylaspartate (NAA), choline-containing compounds (Cho) and creatine plus phosphocreatine (Cr). In addition more complex peaks can be identified from protons in a range of metabolites, if present, including lactate, inositols (specifically myo-inositol (mI)), alanine, glutamine (Gln) and glutamate (Glu).
Physiological changes in the 1H MR spectrum occur during the first 2–3 years of postnatal life with the most important changes occurring in the first year. Age-specific, reproducible, regional studies with absolute quantitation of 1H MR metabolites are difficult to obtain73. Adult norms are more established and are achieved by 3 years of age, reflecting the successful completion of most myelination and organization events.
N-acetylaspartate (NAA)
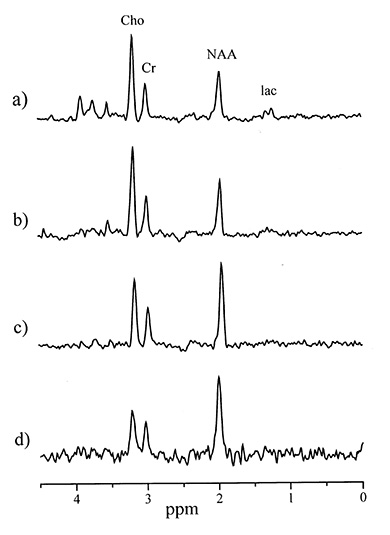
Fig. 16.3 1H MR spectra (TE 270 ms) from an 8 cm3 voxel within the basal ganglia of (a) a normal preterm infant (GA 29+3 weeks (age at scan 35+1 weeks)), (b) a normal preterm infant, (c) an infant aged 6 months with normal neurodevelopmental outcome, and (d) an adult control. This series demonstrates that by 6 months NAA has become the dominant peak in the spectrum, the Cho/Cr ratio decreases with maturation and that lactate is only easily visible in the preterm infant.
All studies have documented an increase in NAA after birth, with it becoming the dominant peak by 6 months of age51, 53, 103 (Fig. 16.3). Quantification studies have confirmed an almost doubling of NAA from birth levels of 4.82 mM to adult levels of 8.89 mM53.
NAA is an amino acid found exclusively in the nervous system and is synthesized in brain mitochondria from acetyl-CoA and aspartate by the enzyme l-aspartate N-acetyl transferase. NAA has been used as a neuronal marker, as apart from oligodendrocyte type 2 astrocyte precursors, NAA is present primarily in neurons102. More recently, studies have demonstrated that mature oligodendrocytes can express NAA in vitro when trophic factors are used6; this observation challenges the concept that NAA changes observed in vivo 1H MRS reflect neuronal function alone. The exact functions and roles of this amino acid are unclear although it is thought to have a role in myelination in the developing brain, metabolism of specific brain fatty acids, ion balance and neuromodulation. NAA can be detected in the cerebral cortex and white matter of fetuses as early as 16 weeks’ gestation and shows an age-dependent increase in synthetic rate which is reflected in total brain levels of NAA49.
Using in vivo 1H MRS and high performance liquid chromatography, a marked regional variation in NAA concentrations was demonstrated in healthy preterm (gestational age (GA) 27–34 weeks studied at postconceptional age (PCA) 31–37 weeks) and term infants with higher concentrations in the thalamus and basal ganglia than the frontal lobe and precentral gyrus47. Of note, the NAA concentrations of these healthy preterm infants at 40 weeks PCA were no different to NAA concentrations of term newborns studied shortly after birth.
TABLE 16.3 Absolute concentrations of different age groups in mmol/kg brain tissue (mean +/ñ1 SEM) and significance tests for the differences found. (Reproduced with permission of Wiley from Kreis et al.53)
Group | n (ROIs) | GA (weeks) | pn age (weeks) | NAA | Cr | Cho | ml |
---|---|---|---|---|---|---|---|
<42GA | 11 | 38.7 ± 0.7 | 3.9 ± 1.6 | 4.82 ± 0.54a | 6.33 ± 0.32 | 2.41 ± 0.11 | 10.1 ± 1.1 |
42–60GA | 8 | 50.2 ± 1.8 | 9.3 ± 1.9 | 7.03 ± 0.41 | 7.28 ± 0.28 | 2.23 ± 0.06 | 8.52 ± 0.92 |
<2pn | 6 | 40.0 ± 1.0 | 0.8 ± 0.2 | 5.52 ± 0.64 | 6.74 ± 0.43 | 2.53 ± 0.12 | 12.4 ± 1.4 |
2–10pn | 7 | 41.9 ± 1.5 | 3.8 ± 0.9 | 5.89 ± 0.21 | 6.56 ± 0.44 | 2.16 ± 0.09 | 7.69 ± 0.62 |
Adult | 10 | 1440 ± 68 | 8.89 ± 0.17 | 7.49 ± 0.12 | 1.32 ± 0.07 | 6.56 ± 0.43 | |
p value: 42GA versus adult | <0.0001 | <0.0001 | <0.0001 | 0.001 | |||
p value: 42GA versus 42 to 60GA | 0.0007 | 0.02 | 0.07 | 0.10 | |||
p value: 42 to 60GA versus adult | <0.0001 | 0.16 | <0.0001 | 0.0003 | |||
p value: <2pn versus adult | <0.0001 | 0.005 | <0.0001 | <0.0001 | |||
p value: <2pn versus 2 to 10pn | 0.7 | 0.8 | 0.02 | 0.004 | |||
p value: 2–10pn versus adult | <0.0001 | 0.001 | <0.0001 | 0.007 | |||
aMinima and maxima are highlighted in bold. ROI, region of interest; pn, postnatal; GA, gestational age; NAA, N-acetyl aspartate; Cr, creatine; Cho, choline; | |||||||
mI, myo-inositol. |
< prev | top | contents | next >
Choline-containing compounds (Cho)
The Cho peak includes free choline, phosphocholine and glycerophosphocholine. These compounds are intermediates in phospholipid metabolism. The Cho/Cr ratio decreases with increasing postnatal age until about 3 years of age. Quantification studies confirm that the newborn brain contains higher concentrations of Cho than the adult brain53. The reduction in Cho/Cr mirrors the fall in PME with age and it has been suggested that the accelerated myelination during the first few months of life incorporates a proportion of these ‘NMR-visible’ choline residues into an ‘invisible’ macromolecule associated with myelin53. Cho/Cr is high in the acute state of demyelinating disease and some infants with neurologic abnormalities: this may represent the inverse process described above or an abnormality in phospholipid metabolism.
Creatine plus phosphocreatine (Cr)
The equilibrium enzyme creatine kinase converts Cr to PCr, which is the short-term energy storage form of the tissue. The total measured Cr (sum of PCr and Cr), therefore, may reflect the energy potential available. As seen from Table 16.3, the concentration of Cr increases rapidly before and around term53.
Regional variation and differing rates of increase in Cr concentrations have been demonstrated in healthy preterm infants. At term, Cr is significantly higher in the thalamus and basal ganglia compared to the frontal lobe and precentral gyrus47. These findings are in concordance with positron emission tomography studies showing local cerebral metabolic rates for glucose to be highest in the thalamus and basal ganglia22.
Several MRS studies of term infants after hypoxic injury (HI) have used Cr as a metabolite of reference because the Cr concentration has been reported to be relatively stable after such an insult18.
Studies in neonatal rats have explored whether systemic injections of creatine are neuroprotective – in one study creatine injection 3 days prior to a hypoxic insult increased survival and suppressed seizures in the immature rat45. Further studies are needed.
Lactate
The doublet signal at 1.33 ppm is easily observed when cerebral lactate levels are increased, although good spatial localization is required to eliminate interfering resonances from extracranial lipids. Confrmation of the assignment of the lactate doublet resonance is readily established by varying the echo time (TE 135 ms doublet inverted, TE 270 ms doublet upright) (Fig. 16.4) .
Lactate and its redox partner pyruvate have a central position as the terminal metabolites of glycolysis within the cytosol and pyruvate as the initial substrate for the mitochondrial tricarboxylic acid (TCA) cycle. The lactate concentration must rise whenever the glycolytic rate in a volume of tissue exceeds the capacity to catabolize lactate or export it to the bloodstream. The prospect of measuring cerebral lactate was one of the driving forces for the development of localized in vivo 1H MRS because of its importance as a marker of tissue ischemia and hypoxia. During normal aerobic conditions, lactate is present in the normal adult brain at concentrations of approximately 0.3 to 1 mM, which is close to the NMR detectable limit. In hypoxia and stroke brain lactate can increase to concentrations above 10 mM. Lactate can be detected in the brain of healthy preterm infants; indeed studies estimate brain lactate concentrations to be 0.9–2.4 mM in this patient group, the concentration increasing with decreasing gestational age and degree of growth restriction57. This may be a reflection of the immature stage of enzyme development in the preterm brain9, 85 and is explained by the increasing dependence on glycolysis compared with mitochondrial respiration with immaturity. This less effcient method of energy production may be suffcient for the preterm brain as the energy requirement (measured by oxygen uptake) is low at 26 to 32 weeks for example, when it is around one sixth that of the adult brain and one half of the term brain1. Regional variation in lactate ratios have been demonstrated with higher concentrations seen in the occipitoparietal regions compared to the basal ganglia suggesting a relative immaturity and a reduction in oxidative phosphoylation in this particularly vulnerable area79.
For some time it has been known that plasma lactate can be used as a fuel in the normal preweaning neonatal brain104; studies on monocarboxylate transporters have clarifed this further. These transporters, present on the blood-brain barrier in early life, transport lactate from plasma to brain, however after weaning these transporters disappear and plasma lactate can no longer be used as a major fuel by the brain76.
Although plasma lactate cannot substitute for glucose as a metabolic substrate in the post weaning brain because of its limited permeability across the blood brain barrier, it is now clear that in physiological states lactate formed within the brain parenchyma (for example through glutamate-activated glycolysis in astrocytes) can fulfill the energetic needs of neurons. Indeed in vivo 1H MRS studies in humans demonstrate a transient lactate peak in the primary visual cortex during physiological stimulation; this is consistent with neuronal activation-induced glycolysis84. The following mechanism for glutamate-induced glycolysis in astrocytes during physiological activation has been proposed and confrmed by in vitro experimental evidence77, 78 (Fig. 16.5).
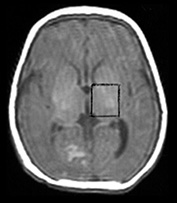
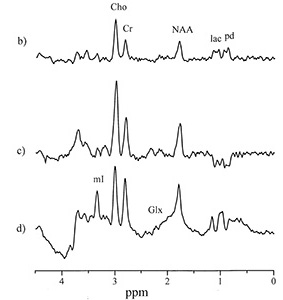
Fig. 16.4 Male term infant aged 5 days following neonatal encephalopathy due to birth asphyxia. (a) T1 weighted spin echo transverse image (SE 500/20) at the level of the basal ganglia. There is some abnormal signal intensity in the thalami and the posterior lentiform nucleus on the right. There is a diminished signal from myelin in the posterior limb of the internal capsule. (b,c,d)1H MR spectra (PRESS) from a 2cm3 voxel encompassing the left basal ganglia in the same infant with neonatal encephalopathy demonstrating modulation of lactate doublet at various echo times, (b) the lactate doublet is upright at TE 270ms, (c) the lactate doublet is inverted at TE 135ms, and (d) the lactate doublet is out of phase at TE 40ms. This modulation pattern is determined by the 7Hz coupling constant of the lactate doublet. Propan-1,2-diol, the carrier for phenobarbital and phenytoin, resonates at 1.1ppm and also undergoes phase modulation at different echo times.
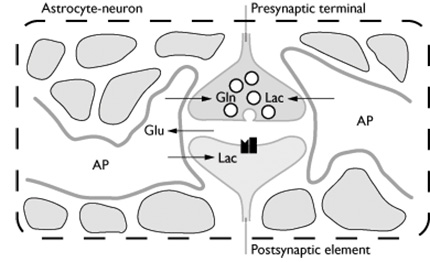
Fig. 16.5 The astrocyte-neuron metabolic unit. Glutaminergic terminals and the astrocytic processes that surround them can be viewed as a highly specialized metabolic unit in which the activation signal (glutamate) is manufactured by the neuron and presented to the astrocyte. Glutamate is then taken up by the astrocyte, thus removing glutamate from the extracellular space (ECS) and protecting surrounding cells from excitotoxicity from glutamate. Glutamate taken up by the astrocyte is converted to glutamine, then released into the ECS where it is taken up by neurons and converted back to glutamate for further signaling. In the astrocyte, meanwhile, glutamate stimulates glucose uptake and glycolysis to lactate. The lactate is then ‘shuttled’ to the neuron where it can be used as a fuel (Reproduced with permission of the Academic Press from Zigmond et al113.)
Glial cells make up approximately half of the brain volume and the astrocyte is one of the predominant glial cells. Astrocytes outnumber neurons by as much as 10:1 even in the healthy brain and are ideally suited to couple local changes in neuronal activity with adaptations in energy metabolism. Astrocytic perivascular endfeet surround brain capillaries; this therefore is a likely site for the predominant uptake of glucose. In addition astrocytes have processes which ensheathe synaptic contacts. Neuronal activity leads to release of glutamate, which depolarizes postsynaptic neurons. The action of glutamate is terminated by an effcient glutamate uptake system located in astrocytes. Glutamate is co-transported with Na+, leading to an increase in the intra-astrocytic concentration of Na+, which in turn leads to an activation of the astrocyte Na+, K+-ATPase. Activation of the Na+, K+-ATPase stimulates glycolysis (glucose utilization and lactate production). Lactate is then released into the extracellular space to be used by neurons.
myo-Inositol

Fig. 16.6 1H MR spectra (TE 40ms) from an 8cm3 voxel within the basal ganglia of (a) a normal preterm infant (GA 29+3 weeks (age at scan 35+1 weeks)), (b) a normal term infant, (c) an infant aged 6 months with normal neurodevelopmental outcome, and (d) an adult control. This series demonstrates the added metabolite information obtained at short TE time, particularly from myo-inositol and glutamine/glutamate. Myo-Inositol is prominent in the spectra from the preterm and newborn infants.
myo-inositol is a naturally occurring sugar and is normally present at about 5mm in the adult brain. It can be visualized by 1H MRS at short echo times (keeping T2 and J-coupling effects to a minimum). One resonance can be observed at approximately 3.56ppm (Fig. 16.6) and a second distinct resonance of myo-inositol at 4.06ppm, however this is too close to the partially suppressed water peak to be reliably quantified. Seventy per cent of the major myo-inositol resonance is myo-inositol itself, 15% myo-inositol-monophosphate, and 15% glycine94.
myo-Inositol is one of the dominant peaks at short TEs in newborn proton spectra (Fig. 16.6). The intracerebral concentration of myo-inositol at birth is approximately 12mm and it decreases rapidly after birth to reach adult levels at around 2 months. Although there is a trend toward a higher myo-inositol concentration the more premature the baby, the concentration of myo-inositol is mostly determined by postnatal age51. This agrees with reports of newborn CSF having 20 times the concentration of myo-inositol than adult CSF and being twice as high in umbilical artery blood than adult plasma. There is evidence to suggest that myo-inositol is associated with surfactant production – administration of myo-inositol to immature animals increased levels of pulmonary surfactant2 and supplementation with myo-inositol in preterm infants reduced severity of respiratory distress syndrome, although exogenous natural surfactant had a more striking effect40.
In 1984, Berridge discovered the activity for which inositols are now best known5 – as an intermediate in the cerebral inositol polyphosphate (IPP) cascade, they are active in releasing Ca2+ from endoplasmic reticulum and mitochondria, thus linking hormone receptor binding with intracellular activity. The inositols therefore are second messengers for the hormone actions of vasopressin, thyrotrophin and angiotensin II for example. myo-Inositol also functions as a major non-nitrogenous osmoregulator in the CNS94. Clinical evidence for such a function is a reversible increase in brain myo-inositol detected with 1H MRS in an infant with severe hypernatremia56 and a decrease in myo-inositol in several patients with severe hyponatremia105 (Fig. 16.7). Other roles proposed for myo-inositol are cell nutrition46 and detoxification52. The myo-inositol peak visible by MR represents free myo-inositol acting as an intracellular osmolyte as myo-inositol involved in signal transduction is mostly bound and present in very small concentration.
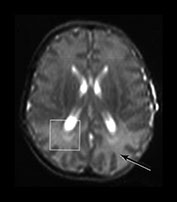

Fig. 16.7 This male infant was born at 23 weeks’ gestation and scanned at 36 weeks. He had severe respiratory distress syndrome and was ventilator dependent for several weeks. One week prior to the scan he developed diarrhea and became dehydrated. His serum sodium increased to 179mmol/l 2 days prior to the scan and was still elevated on the day of scan. (a) T2 weighted images show slightly high signal intensity (arrow) in the posterior white matter bilaterally. (b) 1H MRS spectrum acquired from an 8cm3 voxel in the posterior periventricular white matter demonstrating a raised myo-inositol/Cr of 3.63. This increase in myo-inositol is likely to be in response to the hypernatremia.
There is no consensus on the metabolic significance of an increased or decreased concentration of brain myo-inositol. Increased ratios of cerebral mI have been described in Alzheimer’s disease68, 86, Down’s syndrome97 (Fig. 16.8) and HIV dementia20. Decreased levels of myo-inositol have been described in hepatic encephalopathy52. Possible mechanisms for an increase in myo-inositol are: (i) loss of neurons and replacement by glia (gliosis) as this might result in a reciprocal loss of NAA and increase in myo-inositol/Cr. This presumption rests on the in vitro work by Brand et al. on embryonic nervous tissue in culture which identified myo-inositol as a marker for glia11 although other more recent in vitro studies have documented high levels of myo-inositol in neuronal cultures70; and (ii) osmotic stress, which may upregulate the expression of the sodium/myo-inositol cotransporter (SMIT), thereby increasing intracellular myo-inositol levels. Evidence for this comes from in vitro work where the abundance of SMIT mRNA and the transcription rate of the SMIT gene were increased when brain glial cells were cultured in hypertonic medium33. Upregulation of SMIT has been demonstrated in cultured fibroblasts in Down’s syndrome; this may explain the increased myo-inositol seen in these patients (Fig. 16.8).
We have observed changes in myo-Inositol during the first week after birth in infants with severe neonatal encephalopathy. This will be discussed in the section below.


Fig. 16.8 Term-born infant with Down’s syndrome. (a) T2 weighted images show some slightly high signal intensity (arrow) throughout the white matter only. The head is brachycephalic. (b) 1H MRS spectrum (TE 40ms) acquired from the same infant demonstrating a raised myo-inositol/Cr of 1.71.
Glutamate, glutamine and γ amino butyric acid (GABA)
Glutamate is the major neurotransmitter for brain excitatory function and GABA is the major neurotransmitter for inhibitory function. Both of these neurotransmitters are taken up by the surrounding glial cells after release from the nerve terminal. Glutamate re-uptake and synthesis is required or else the nerve terminal precursor pool will become depleted. Glial cells are able to transport glutamate from the synaptic cleft so maintaining low concentrations of extracellular glutamate. Glutamate taken up by glia is converted to glutamine by glutamine synthetase, an enzyme exclusively in glia. Glutamine is released from the glia to the ECF where it is taken up by neurons and converted back to glutamate. The localization of glutamate and glutamine as well as the enzyme glutamine synthetase provides the basis for studying neuronal/glial interactions in vivo by MRS. Carbon-13 (13C) MRS has been used to obtain information about glial and neuronal metabolism as well as metabolic flux, enzyme activities and metabolic regulation in vivo of the 13C isotopic enrichment of specific carbon positions of glutamate and glutamine.
< prev | top | contents | next >
Cerebral pathology
In the following section 31P and 1H MRS findings are discussed with reference to term neonatal encephalopathy and preterm brain injury. Recently considerable doubt has been cast on the assumption that perinatal hypoxia-ischemia (HI) is the primary cause of neonatal encephalopathy and cerebral palsy. Important epidemiological studies have suggested a wide range of alternative causal pathways such as maternal fever during labor, chorioamnionitis, maternal thyroid disease and abnormalities of the haemostatic system. There appears, however, to be at least a subgroup of infants with neonatal encephalopathy who have cerebral metabolic changes entirely characteristic of acute cerebral hypoxia–ischemia in the perinatal period.
Damage to the preterm brain is an increasingly important problem as more extremely low birth weight infants are surviving. Less is known about the cerebral metabolic events associated with preterm brain injury; however, there is increasing evidence of a major role of infection or inflammatory processes while secondary energy failure is unlikely to have a central role.
TERM NEONATAL ENCEPHALOPATHY AND FOLLOW-UP
Secondary energy failure
ATP is the energy currency for the cell. In situations where oxidative phosphorylation is impaired, such as hypoxia– ischemia, [ATP] will fall; the fall is initially extremely small because of the buffering effect of the creatine kinase reaction. While this reaction maintains [ATP] close to normal, [PCr] falls and [Pi] increases, that is the PCr/Pi ratio decreases. This ratio is proportional to the phosphorylation potential of the tissue and is a measure of the energy reserve of the tissue. Only when [PCr] becomes depleted to lmm does the [ATP] fall.
31P MRS has been important in demonstrating the biphasic pattern of energy failure during and after hypoxia–ischemia. Experimental studies in several species of mammal have confirmed this biphasic pattern of cerebral metabolic abnormality during and after cerebral hypoxia–ischemia. In the piglet during global hypoxia–ischemia59,80 and during focal stroke in rat pups7,108 there was ‘primary’ energy failure: intracerebral PCr/Pi fell and the pHi became acidotic. Eventually [ATP] fell, but on resuscitation, metabolites returned to normal within 1–2h. Some 6–12 hours later, the period of secondary energy failure began during which PCr/Pi again declined, lactate increased and the pHi became alkaline. There was a dose response between the severity of HI insult and the magnitude of the secondary changes in cerebral energy metabolism. The more severe the cerebral metabolic injuries, the worse the histological injury.
MRS of term infants with neonatal encephalopathy demonstrated identical abnormalities of cerebral energy metabolism. The primary event cannot be observed but newborn infants following HI were seen to have normal phosphorylation potential and pHi on the first day of life. 8 to 24h after birth a delayed decline in PCr/Pi or ‘secondary’ energy failure occurred4 (Fig. 16.9) accompanied by an alkalotic pHi and an increase in brain lactate43. The magnitude of PCr/Pi drop during secondary energy failure was seen to correlate with subsequent neurodevelopmental abnormality and reduced cranial growth 1 and 4 years later95, 96. Elevated cerebral lactate within 18h of birth asphyxia predicted adverse outcome at 1 year of age43 (Fig. 16.10 and Fig. 16.11). Recent data from our laboratory suggest that brain myo-inositol/Cr is increased during the period of secondary energy failure in those infants with neonatal encephalopathy associated with increased lactate/Cr, MRI changes of severe injury and a poor neurodevelopmental outcome at one year. A possible explanation for this acute change in myo-inositol/Cr may be the induction of the sodium/myo-inositol cotransporter (SMIT), one of the many gene expressions to be influenced by an acute insult109, 110.

Fig. 16.9 31P spectra from a birth-asphyxiated infant born at 37 weeks’ gestation: postnatal ages at the time of study are indicated. At 8h PCr/Pi was 0.99, ATP/total P was 0.09, and pHi was 7.06: pHi rose to a maximum of 7.28 at 36h. Minimum value for PCr/Pi was 0.32 at 55h when ATP/total P was 0.04 and pHi 6.99. The infant died at 60h. (Reproduced with permission of the International Pediatric Research Foundation, Inc., from Azzopardi et al.3.)
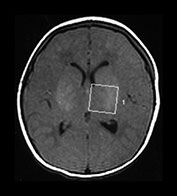


Fig. 16.10 Female term infant with severe birth asphyxia imaged at 2 days of age. MR data obtained on a Picker Eclipse system. (a) A T1 weighted spin echo sequence (SE 500/20) in the transverse plane at the level of the basal ganglia. The brain is normally formed although edematous. There is abnormal high signal intensity in the lentiform and thalami nuclei and an absence of signal from myelin in the posterior limb of the internal capsule. There is loss of gray/white matter differentiation (b) 31P MR spectrum taken from a 125cm3 voxel encompassing the whole brain in the same baby demonstrating a depletion of ATP and PCr, a markedly raised Pi and an alkaline pHi consistent with an abnormal outcome with severe neurodevelopmental impairment. (c) 1H MR spectrum from an 8cm3 voxel (left basal ganglia) in the same baby demonstrating a decreased NAA and markedly increased lactate levels. The MR findings are associated with an abnormal outcome with severe neurodevelopmental impairment and this baby died on day 5.

Fig. 16.11 Medians, interquartile ranges, 5th and 95th centiles of lactate/Cr measured by 1H MRS during the first 18h after birth in asphyxiated infants in whom later measurements found PCr/Pi <0.75 (severe) or PCr/Pi >0.75 (mild) and in controls (control). Values in the three groups are significantly different (Kruskall-Wallis). (Reproduced with permission of MacKeith Press from Hanrahan et al.43.)
Follow-up after neonatal encephalopathy
Lactate can persist in the brain for weeks or months in infants who develop neurodevelopmental impairment (Fig. 16.12). Lactate was detected in the brain using 1H MRS after 4 weeks of age in seven out of eight infants with abnormal neurodevelopmental outcome at 1 year after perinatal asphyxia; none of the infants with normal outcome at 1 year showed evidence of persisting lactate42. A recent study of 43 babies following perinatal asphyxia demonstrated a significant relationship between persisting increased cerebral lactate levels and alkaline pHi, that is a persisting lactic alkalosis (Fig. 16.13) and also decreased phosphorylation potential (increased Pi/PCr)89. Comparing those infants with normal or abnormal neurodevelopmental outcomes at 1 year of age, there were significant differences for lactate/Cr measured at most times, pHi measured at all times, Pi/PCr measured in the first 2 weeks and NAA/Cr measured after the first 2 weeks. pHi was the strongest predictor of outcome at all times up to 30 weeks postnatal age.

Fig. 16.12 Representative 1H MR spectra from the basal ganglia of a term infant with severe birth asphyxia. The 1H MR data were collected using chemical shift imaging techniques with TE 130ms. A signal from lactate could be detected at all the time points studied. The inset 31P MR spectrum at 2 days of age showed severe secondary energy failure, consistent with an abnormal outcome.


Fig. 16.13 Lactate/Cr plotted against pHi for normal (a) and abnormal (b) outcome. Linear regression analysis showed a significant relationship between lactate/Cr and pHi. The relationship was the same irrespective of outcome. (Reproduced with permission of the International Pediatric Research Foundation, Inc. from Robertson et al.89.)
Possible causes for this persisting cerebral intracellular lactic alkalosis are: (i) an altered redox state caused by an inability to regenerate NAD+; (ii) infiltration of activated phagocytes which have an enhanced glycolytic rate thus increasing lactate production58; (iii) altered buffering mechanisms (e.g. Na+/H+ antiporter) in the remaining cells32 and/or (iv) stimulation of glycolysis in the astrocyte with production of lactate which can then be used as a fuel for neurons. Glutamate uptake is known to stimulate glycolysis, similarly an alkaline milieu inside the cell will stimulate glycolysis due to the pH dependency of the glycolytic enzyme phosphofructokinase. Further understanding of the mechanisms leading to these prolonged changes may lead to other modalities of intervention with the aim of improving neurodevelopmental outcome88.
We found a significantly lower NAA/Cr in infants with an abnormal outcome at 1 year after neonatal encephalopathy after 2 weeks of age89. Other studies have shown a reduced NAA/Cr at 7 days37, 14 days75 and at 3 months37 and this has been shown to be indicative of an abnormal outcome after perinatal HI. A further study done in infants at an earlier stage after birth asphyxia (median of 1.3 days)79 showed no convincing reduction with age-matched controls, however in some very severely damaged infants we have demonstrated a reduction in NAA/Cr at <24h.
In summary, 1H and 31P MRS have been important in furthering our understanding of the metabolic response to injury of the brain in the term infant and have led us to suspect an on-going metabolic derangement following HI in those with abnormal neurodevelopmental outcome.
PRETERM BRAIN INJURY
The more subtle effects of prematurity in the absence of detectable brain injury are now well recognized and include minor neurocognitive impairments, behavioral difficulties and specific learning disorders. It is unknown whether prematurity itself affects brain development with data suggesting either delay, acceleration or no effect. A comparison of ex-preterm infants (mean GA 30.5 +/– 1.8 weeks) at a corrected age of term with term-born infants using 1H MRS revealed no statistically significant differences in concentrations of NAA, Cho and myo-inositol between groups48, although there were differences in imaging findings and in some behavior parameters.
It is well known that structural brain injury occurring in preterm infants, such as periventricular leukomalacia (PVL) and hemorrhagic parenchymal infarction (HPI), have an impact on later cognitive and neuromotor performance106. It is now generally accepted that white matter damage (WMD) in the premature infant is a better predictor of poor neurologic outcome than isolated intraventricular hemorrhage (IVH)74 and that IVH with ventricular dilatation are powerful predictors of WMD occurrence55. In a 2-year cohort study of infants born at <30 weeks’ gestation there was a high incidence of MRI abnormality in the white matter (WM)60. This was associated with high concentrations of pro-inflammatory cytokines and T-cell activation in umbilical cord blood, which accords well with other data suggesting that fetoplacental infection is a cause of both preterm birth and cerebral WM injury25. There are at present no MRS studies looking at the characteristics of this WM.
White Matter Damage (WMD)
We have recently assessed the biochemical characteristics of WMD (consisting of both PVL and HPI) using 1H and 31P MRS and compared this to preterm infants with no WMD at a corrected age of term. 30 infants (gestational age 27.9 ± 3.1 weeks, birth weight 1122 ± 4459) were studied at a postnatal age of 9.8 ± 4.1 weeks (corrected age 40.3 ± 3.9 weeks92). In the WMD group, mean lactate/Cr and myo-inositol/Cr were higher than the normal WM group. There was no difference in the NAA/Cr, Cho/Cr or pHi between the two groups. We found the absence of an alkaline pHi in cases with a markedly elevated brain lactate interesting; the relationship between pHi and lactate/Cr appears to differ in preterm infants compared to term infants following neonatal encephalopathy. For a given lactate/Cr in a preterm infants the pHi was never as alkaline as it was in term infants after perinatal HI. Both the type of injury and response of the extremely preterm brain to an insult may differ from that in the term brain (Fig. 16.14).
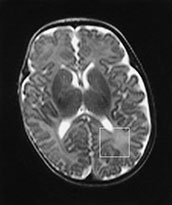
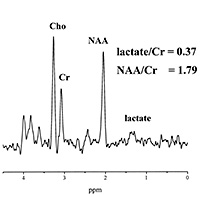

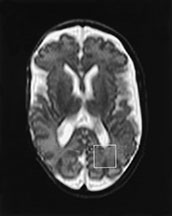
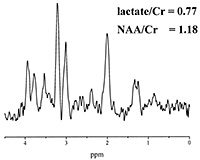
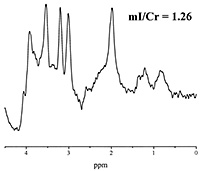
Fig. 16.14 (a) T2-weighted magnetic resonance (MR) image and the 8-cm3 voxel localized to the posterior periventricular white matter (WM) from where MRS data were obtained from an infant born at 24 weeks gestation and scanned at 46 weeks corrected age with normal WM on MRI. The MRS data acquired using (b) echo time (TE) of 270 milliseconds and (c) TE of 40 milliseconds from the posterior periventricular WM are shown. (d) T2-weighted MR image gestationa nd the 8-cm3 voxel localized to the posterior periventricular WM from where MRS data were obtained from an infant born at 26 weeks gestation and scanned at 36 weeks corrected age. The MRI demostrated multiple abnormal high signal intensity lesions in the white matter and widespread atrophy. The MRS data acquired using (e) TE of 270 milliseconds and (f) TE of 40 milliseconds from the posterior periventricular WM are shown. These spectra demonstrate an increased lactate/Cr and myo-inositol/Cr in the infant with WMD (e and f) compared with those from the infant with the normal MRI (b and c); these differences were significant between groups even when age of scan was included in the analysis. Although the NAA/Cr in the infant with white matter damage (e) was less than the infant with normal WM(b), this difference was not significant when all infants in each group were compared and age of scan was included in analysis. Cr, creatine plus prosphocreatine; Cho, choline-containing compounds; NAA, N-acetyl aspartate; Glx, glutamine/glutamate. (Reproduced with permission of Lipincott Williams and Wilkins, inc. from Robertston et al92.)
Periventricular leukomalacia (PVL)
Few studies have looked at the preterm brain with PVL. Hyperechoic areas (‘flares’ or echo densities) on cerebral ultrasound are thought to reflect disturbances in the periventricular blood circulation, resulting in some cases, in local destruction of tissue and development of cysts in the periventricular white matter which are in turn demonstrable as hypoechoic areas (cysts), consistent with periventricular leukomalacia26. Many of the hyperechoic regions seen on ultrasound will disappear and leave no visible trace on ultrasound. 31P MRS was used to study 27 preterm and term infants with increased echo densities on cranial ultrasound in the first few days of life and compared these findings to 18 normal infants. This showed that the PCr/Pi ratio was predictive of outcome41.
Groenendaal et al.38 used localized PRESS 1H MRS to study preterm infants with PVL and term infants with subcortical leukomalacia. A low NAA/Cho predicted poor outcome although some infants developed unfavorably despite a normal NAA/Cho ratio. The absence or presence of lactate did not improve prediction of outcome. Failure of 1H MRS to accurately predict outcome may be due to partial volume effects or difficulties with localization.
Intraventricular hemorrhage (IVH)
A small number of studies have been done in preterm infants with IVH. Two studies are discussed here. High energy
phosphorus metabolites and pHi were measured in five babies with grade 3 to 4 IVH and compared to 15 preterm infants without IVH112. The group of babies with the IVH had significantly lower estimated gestational age (GA) (mean 26.8 versus 30.0 weeks), 5min Apgar score and required a longer period of ventilation and had lower PCr/Pi and PCr/ATP ratios than those without an IVH.
1H MRS was used to investigate the metabolic consequences of germinal matrix hemorrhage in the striatal region of 12 preterm infants of GA mean (SD) 29.3 +/– 2.5 weeks101. Both sides were studied twice and in most infants the hemorrhage was unilateral or larger on one side: the side with the (larger) hemorrhage was compared to the less-affected side. The findings were that germinal matrix hemorrhage (GMH) was initially followed by lactate accumulation and a presumed delay in maturation as indicated by transiently low Cr and NAA indices. At a corrected age of 3months, there was no difference in these metabolite indices; however, there was an increase in the Cho index suggesting a more persistent metabolic change after GMH.
< prev | top | contents | next >
Conclusions
This review has illustrated that a wide range of MR spectral changes are observed in neonatal pathologies. After HI, lactate/Cr and myo-inositol/Cr may be markedly elevated, PCr/Pi dramatically reduced and pHi alkaline. Changes in lactate/Cr and myo-inositol/Cr have been observed in prematurity. For all these studies, knowledge of age-related normative ranges are essential for spectral interpretation. However, data from normal subjects are difficult to obtain, not only because of a general reluctance to examine healthy infants but also because they do not keep still enough for long.
MRS has particularly contributed to the understanding of brain injury after HI, in illustrating the concept of delayed cerebral energy failure, in defining a possible ‘therapeutic window’ some hours after the insult when intervention may ameliorate brain injury, and also in contributing to the concept that there are persisting metabolic changes in the brain months after HI. Combined with laboratory and cell work, these findings may aid in the understanding of mechanisms leading to these biochemical changes.
Exploiting the non-invasive nature of multinuclear MRS, a major advantage of the technique is that longitudinal studies can be readily achieved enabling insights into how pathophysiological processes evolve in vivo. It may also be used to assess the effectiveness of treatment regimens.
One of the major challenges in clinical in vivo MRS is the necessity to quantify accurately metabolite concentrations within the timescale of a neonatal examination. It continues to be difficult to obtain enough measurements to interpret a spectral peak in terms of a metabolite concentration rather than a metabolite ratio. Improvements in technical methodologies are central to further developments in clinical MRS. For example, chemical MRS studies have benefited from the use of higher magnetic fields, and the same may be expected for clinical MRS studies. A whole-body MR system based on an 8 T magnet is available93, and 3 T systems are becoming more widely available for clinical use. Better resolution from smaller voxel sizes and improved editing of artifacts can be expected in the future; for example, improved definition of spectral changes due to voluntary or involuntary movements72.
Other recently developed 1H MRS techniques take advantage of the interaction of the proton nucleus with other nuclei such as the 13C and 15N nuclei. Studies of glucose and amino acid metabolism in the human brain have been performed using 13C-labeled glucose permitting measurements of Kreb’s cycle, glutamine synthesis and glycolytic fluxes in vivo63. This technique is likely to be useful in understanding mechanisms of neurologic disorders in infants.
In summary, MRS studies can provide a non-invasive insight into in vivo brain biochemistry. Combined with newer MRI techniques such as diffusion weighted imaging enabling visualization of tracts, MRS can be expected to define brain injury in the neonate with more precision.
< prev | top | contents | next >
Summary
- Nuclear magnetic resonance spectroscopy is one of the most important tools for quantitative analysis of chemical composition and structure, and this technique is now being applied in vivo to study biochemical process in the term and preterm infant.
- Interpretation of a clinical MR spectrum can provide information about cellular energetics, membrane turnover, neuronal function, selected neurotransmitter activity and intracellular pH.
- Proton MR spectra can be acquired using a standard MRI system with appropriate sequences but the static magnetic field should be at least 1 Tesla to achieve adequate sensitivity. For other nuclei a multinuclear radiofrequency capability is required.
- An understanding of age-dependent and regional changes in metabolite ratios and absolute concentrations of 31P and 1H metabolites is important to enable accurate identification and recognition of metabolite abnormalities in pathological states.
- Seven main peaks can be assigned in a cerebral phosphorus spectrum to phosphomonoesters (PME), inorganic phosphate (Pi), phosphocreatine (PCr), phosphodiesters (PDE) and the three phosphate groups (a, b, g) in nucleoside triphosphate (NTP).
- Three singlet peaks can be readily assigned in the proton spectrum to N-acetylaspartate (NAA), choline-containing compounds (Cho) and creatine plus phosphocreatine (Cr). In addition more complex peaks can be identified from protons in a range of metabolites, if present, including lactate, inositols (specifically myo-inositol), alanine, glutamine (Gln) and glutamate (Glu).
- Cerebral 1H and 31P MRS have been important in furthering our understanding of the response to injury of the brain following hypoxia–ischemia. Newborn infants with neonatal encephalopathy show a ‘secondary’ energy failure 8–24h after birth. The magnitude of the spectral abnormalities during secondary energy failure are predictive of adverse outcome at 1 year of age. Increased lactate levels and an alkaline intracellular pH can persist for weeks or months in those infants who develop neurodevelopmental impairment.
- Cerebral 1H and 31P MRS findings are discussed in preterm brain injury, specifically white matter damage, periventricular leukomalacia and intraventricular hemorrhage.
< prev | top | contents | next >
References
- Altman DI, Perlman JM, Volpe JJ (1993) Cerebral oxygen metabolism in newborns. Pediatrics 92: 99–104.
- Anceschi MM, Petrelli A, Zaccardo G et al. (1988) Inositol and glucocorticoid in the development of lung stability in male and female rabbit fetuses. Pediatr Res 24, 617–621.
- Azzopardi D, Wyatt JS, Hamilton PA et al. (1989) Phosphorus metabolites and intracellular pH in the brains of normal and small for gestational age infants investigated by magnetic resonance spectroscopy. Pediatr Res 25, 440–444.
- Azzopardi D, Wyatt JS, Cady EB et al. (1989) Prognosis of newborn infants with hypoxic–ischemic brain injury assessed by phosphorus magnetic resonance spectroscopy. Pediatr Res 25, 445–451.
- Berridge MJ (1993) Inositol triphosphate and calcium signalling. Nature 361, 315–325.
- Bhakoo KK, Pearce D (2000) In vitro expression of N-acetyl aspartate by oligodedrocytes: implications for proton magnetic resonance spectroscopy signal in vivo. J Neurochem 74, 254–62.
- Blumberg RM, Cady EB, Wigglesworth JS et al. Relation between delayed impairment of cerebral energy metabolism and infarction following transient focal hypoxia ischaemia in the developing brain. Exp Brain Research 113, 130–137.
- Boesch C, Gruetter R, Martin E et al. (1989) Variations in the in vivo P-31 MR spectra of the developing human brain during postnatal life. Work in progress. Radiology 172(1), 197–199.
- Booth REG, Patel TB and Clark JB (1980) The development of enzymes of energy metabolism in the brain of precocial (guinea pig) and non-precocial (rat) species. J Neurochem 34, 17–25.
- Bovee W, Canese R, Decorps M et al. (1998) Absolute metabolite quantification by in vivo NMR spectroscopy: IV. Multicentre trial on MRSI localisation tests. Magn Reson Imaging 16(9), 1113–1125.
- Brand A, Richter-Landsberg C and Leibfritz D (1993) Multinuclear NMR studies on the energy metabolism of glial and neuronal cells. Dev Neurosci 15, 289–298.
- Buchli R and Boesiger P (1993) Comparison of methods for the determination of absolute concentrations in human muscles by 31P MRS. Magn Reson Med 30, 552–628.
- Buchli R, Martin E, Boesiger P et al. (1994) Developmental changes of phosphorus metabolite concentrations in the human brain: a 31P magnetic resonance spectroscopy study in vivo. Pediatr Res 35(4), 431–435.
- Cady EB (1995) Quantitative combined phosphorus and proton PRESS of the brains of newborn human infants. Magn Reson Med 33, 557–563.
- Cady EB, Costello AM, Dawson JM et al. (1983) Non-invasive investigation of cerebral metabolism in newborn infants by phosphorus nuclear magnetic resonance spectroscopy. Lancet 8333, 1059–1062.
- Cady EB, Lorek A, Penrice J et al. (1994) Detection of propan-1, 2-diol in neonatal brain by in vivo proton magnetic resonance spectroscopy. Magn Res Med 32, 764–776.
- Cady EB, Penrice J, Amess PN et al. (1996) Lactate, N-acetylaspartate, choline and creatine concentrations, and spin–spin relaxation in thalamic and occipito-parietal regions of developing human brain. Magn Reson Med 36(6), 878–886.
- Cady EB, Amess P, Penrice J et al. (1997) Early cerebral-metabolite quantification in perinatal hypoxic–ischaemic encephalopathy by proton and phosphorus magnetic resonance spectroscopy. Magn Reson Imaging 15(5), 605–611.
- Chang L, Ernst T, Osborn D et al. (1998) Proton spectroscopy in myotonic dystrophy: correlations with CTG repeats. Arch Neurol 55, 305–311.
- Chang L, Ernst T, Leonido-Yee M et al. (1999) Cerebral metabolite abnormalities correlate with clinical severity of HIV-1 cognitive motor complex. Neurology 52(1), 100–108.
- Christiansen P, Toft PB, Gideon P et al. (1994) MR-visible water content in human brain: a proton MRS study. Magn Reson Imaging 12, 1237–1244.
- Chugani HT, Phelps ME and Mazziotta JC (1987) Positron emission tomography study of human brain functional development. Ann Neurol 22, 487–497.
- Corbett RJT, Laptook AR and Nunnally RL (1987) The use of the chemical shift of the phosphomonoester P-31, magnetic resonance peak for the determination of intracellular pH in the brains of neonates. Neurology 37, 1771–1779.
- Cox IJ (1996) Development and applications of in vivo clinical magnetic resonance spectroscopy. Prog Biophys Molec Biol 65, 45–81.
- Dammann O and Leviton A (1997) Maternal intrauterine infection, cytokines and brain damage in the preterm newborn. Pediatr Res 42, 1–8.
- Dammann O and Leviton A (1997) Duration of transient hyperechoic images of white matter in very low birthweight infants; a proposed classification. Dev Med Child Neurol 39, 2–5.
- de Beer R, Van den Boogaart A, Cody E et al. (1998) Absolute metabolite quantification by in vivo NMR spectroscopy: V. Multicentre quantitative data analysis on the overlapping background problem. Magn Reson Imaging 16(9), 1127–1137.
- de Beer R, Barbiroli B, Gobbi G et al. (1998) Absolute metabolite quantification by in vivo NMR spectroscopy: III. Multicentre 1H MRS of the human brain addressed by one and the same data-analysis protocol. Magn Reson Imaging, 16(9), 1107–1111.
- Dobbing J and Sands J (1973) Quantitative growth and development of the human brain. Arch Dis Child 48, 757–767.
- Duc CO, Weber OM, Trabesinger AH et al. (1998) Quantitative 1H MRS of the human brain in vivo based on the stimulation phantom calibration strategy. Magn Reson Med 39, 491–496.
- Ernst T, Kreis R and Ross BD (1993) Absolute quantification of water and metabolites in the human brain. I. Compartments and water. J Magn Reson B102, 1–8.
- Ferimer HN, Kutina KL and LaManna JC (1995) Methyl isobutyl amiloride delays normalization of brain intracellular pH after cardiac arrest in rats. Crit Care Med 23(6), 1106–1111.
- Fruen BR and Lester BR (1990) Down’s syndrome fibroblasts exhibit enhanced ml uptake. [Abstract] Biochem J 270, 119–123.
- Gadian DG and Gadian DG (Eds) (1995) NMR and its Applications to Living Systems, 2nd edn. New York, Oxford University Press, pp. 139–170.
- Garlick PB, Brown TR, Sullivan RH et al. (1993) Observation of a second phosphate pool in the perfused heart by 31P NMR; is this the mitochondrial phosphate? J Mol Cell Cardiol 15, 855–858.
- Glonek T, Kopp SJ, Kot E et al. (1982) P-31 nuclear magnetic resonance analysis of brain: the perchloric acid extract spectrum. J Neurochem 39, 1210–1219.
- Groenendaal F, Veenhoven RH, van der Grond J et al. (1994) Cerebral lactate and N-acetyl-aspartate/choline ratios in asphyxiated full-term neonates demonstrated in vivo using proton magnetic resonance spectroscopy. Pediatr Res 35, 148–151.
- Groenendaal F, van der Grond J, Eken P et al. (1997) Early cerebral proton MRS and neurodevelopmental outcome in infants with cystic leukomalacia. Dev Med Child Neurol 39(6), 373–379.
- Gruwel MLH, Kuzio B, Deslauriers R et al. (1998) Observation of two inorganic phosphate NMR resonances in the perfused hypothermic rat heart. Cryobiology 37, 355–361.
- Hallman M, Bry K, Hoppu K et al. (1992) Inositol supplementation in premature infants with respiratory distress syndrome. N Eng J Med 326, 1233–1239.
- Hamilton PA, Cady EB, Wyatt JS et al. (1986) Impaired energy metabolism in brains of newborn infants with increased cerebral echodensities. Lancet i, 1242–1246.
- Hanrahan D, Cox IJ, Edwards AD et al. (1998) Persistent increases in cerebral lactate concentrations after birth asphyxia. Pediatr Res 44, 304–311.
- Hanrahan D, Cox IJ, Azzopardi D et al. (1999) Relation between proton magnetic resonance spectroscopy within 18 hours of birth asphyxia and neurodevelopmental outcome at one year of age. Dev Med Child Neurol 41, 76–82.
- Hashimoto T, Tayama M, Yoshimoto T et al. (1995) Proton magnetic resonance spectroscopy of brain in congenital myotonic dystrophy. Pediatr Neurol 12(4), 335–340.
- Holtzman D, Togliatti A, Khait I et al. (1998) Creatine increases survival and suppresses seizures in the hypoxic immature rat. Pediatr Res 44(3), 410–414.
- Holub BJ (1992) The nutritional importance of inositol and the phosphoinositides. N Eng J Med 326(19), 1285–1287.
- Hüppi PS, Fusch C, Boesch C et al. (1995) Regional metabolic assessment of human brain during development by proton magnetic resonance spectroscopy in vivo and by high-performance liquid chromatography/gas chromatography in autopsy tissue. Pediatr Res 37, 145–150.
- Hüppi PS, Schuknecht B, Boesch C et al. (1996) Structural and neurobehavioural delay in postnatal brain development of preterm infants. Pediatr Res 39(5), 895–901.
- Kato T, Nishina M, Matsushita K et al. (1997) Neuronal maturation and N-acetyl-l-aspartic acid development in human fetal and child brains. Brain Dev 19, 131–133.
- Keevil SF, Barbiroli B, Brooks JC et al. (1998) Absolute metabolite quantification by in vivo NMR spectroscopy: II. A multicentre trial of protocols for in vivo localised proton studies of human brain. Magn Reson Imaging, 16(9), 1093–1106.
- Kimura H, Fujii Y, Itoh S et al. (1995) Metabolic alterations in the neonate and infant brain during development: evaluation with proton MR spectroscopy. Radiology 194(2), 483–489.
- Kreis R, Ross BD, Farrow NA et al. (1992) Metabolic disorders of the brain in chronic hepatic encephalopathy detected with 1H MRS. Radiology 182, 19–27.
- Kreis R, Ernst T and Ross BD (1993) Development of the human brain: in vivo quantification of metabolite and water content with proton magnetic resonance spectroscopy. Magn Reson Med 30(4), 424–437.
- Kries R, Ernst T and Ross BD (1993) Absolute quantitation of water and metabolites in the human brain.II.Metabolite concentrations. J Magn Reson B102, 9–19.
- Kuban K, Sanocka U, Leviton A et al. (1999) White matter disorders of prematurity: association with intraventricular haemorrhage and ventriculomegaly. J Pediatr 134, 539–546.
- Lee JH, Arcinue E and Ross BD (1994) Brief report: organic osmolytes in the brain of an infant with hypernatraemia. N Eng J Med 331(7), 439–442.
- Leth H, Toft PB, Pryds O et al. (1995) Brain lactate in preterm and growth-retarded neonates. Acta Pediatr 84, 495–499.
- Lopez-Villegas D, Lenkinski RE, Wehrli SL et al. (1995) Lactate production by human monocyte/macrophages determined by proton MR spectroscopy. Magn Res Med 34(1), 32–38.
- Lorek A, Takei Y, Cady EB et al. (1994) Delayed (‘secondary’) cerebral energy failure following acute hypoxia–ischaemia in the newborn piglet: continuous 48-hour studies by 31P magnetic resonance spectroscopy. Pediatr Res 36, 699–706.
- Maalouf EF, Duggan PJ, Rutherford MA et al. (1999) Magnetic resonance imaging of the brain in extremely preterm infants: normal and abnormal findings from birth to term. J Pediatr 135, 351–357.
- 61 MacKay A, Whittall K, Adler J et al. (1994) In vivo visualisation of myelin water in brain by magnetic resonance. Magn Reson Med 31, 673–677.
- Mandel P and Edel-Harth S (1966) Free nucleotides in the rat brain during postnatal development. J Neurochem 13, 591–595.
- Mason GF, Gruetter R, Rothman DL et al. (1995) Simultaneous determination of the rates of the TCA cycle, glucose utilization, a-ketoglutarate/glutamate exchange and glutamine synthesis in human brain by NMR. J Cereb Blood Flow Metab 15, 12–25.
- Mason GF, Chu WJ, Vaughan JT et al. (1998) Evaluation of 31P metabolite differences in human cerebral gray and white matter. Magn Reson Med 39, 346–353.
- Merboldt KD, Bruhn H, Hanicke W et al. (1992) Decrease of glucose in the human visual cortex during photic stimulation. Magn Reson Med 25(1), 187–194.
- Michaelis T, Merboldt KD, Bruhn H et al. (1993) Absolute concentrations of metabolites in the adult human brain in vivo: quantification of localized proton MR spectra. Neuroradiology 187, 219–227.
- Miller AL and Shamban A (1977) A comparison of methods for stopping intermediary metabolism of developing brain. J Neurochem 28, 1327–1334.
- Miller BL, Moats RA, Shonk T et al. (1993) Alzheimer disease: depiction of increased cerebral myo-inositol with proton MR spectroscopy. Radiology 187, 433–437.
- Moon RB and Richards JH (1973) Determination of intracellular pH by 31P magnetic resonance. J Biol Chem 248, 7276–7278.
- Moore GJ, Koch S, Chen G et al. (1999) myo-Inositol is not exclusively glial in the human CNS. [Abstract] Proc Intl Soc Mag Reson Med 7, 33.
- Nioka S, Chance B, Lockard SB et al. (1991) Quantitation of high energy phosphate compounds and metabolic significance in the developing dog brain. Neurol Res 13(1), 33–38.
- Nguyen Q, Clemence M and Ordidge RJ (1998) The use of intelligent re-acquisition to reduce scan time in MRI degraded by motion. [Abstract] 6th Annual meeting of the International Society for Magnetic Resonance in Medicine, 134.
- Novotny E, Ashwal S and Shevell M (1998) Proton magnetic resonance spectroscopy: an emerging technology in pediatric neurology research. Pediatr Res 44(1), 1–10.
- Paneth N (1999) Classifying brain damage in preterm infants.J Pediatr 134(5), 527–529.
- Peden CJ, Rutherford MA, Sargentoni J et al. (1993) Proton spectroscopy of the neonatal brain following hypoxic-ischaemic injury. Dev Med Child Neurol 35, 502–510.
- Pellerin L, Pellegri G, Martin JL, et al. (1998) Expression of monocarboxylate transporter RNAs in mouse brain: support for a district role of lactate as an energy substrate for the neonatal vs adult brain. Proc Natl Acad Sci USA 95: 3990–3995.
- Pellerin L, Magistretti P 1994 Glutamate uptake into astrocytes stimulates aerobic glycolysis: a mechanism coupling neuronal activity to glycose utilization. Proc Natl Acad Sci 91(22): 10625–10629.
- Pellerin L, Pellegri G, Biltar P et al.(1998) Evidence supporting the existance of an activity-dependent astrocyte-neuron lactate shuttle. Dev Neurosci 20(4–5): 291–299.
- Penrice J, Cady E, Lorek A (1996) Proton magnetic resonance spectroscopy of the brain in normal preterm and term infants, and early changes after perinatal hypoxia–ischaemia. Pediatr Res 40, 6–14.
- Penrice J, Lorek A, Cady EB et al. (1997) Proton magnetic resonance spectroscopy of the brain during acute hypoxia-ischemia and delayed cerebral energy failure in the newborn piglet. Pediatr Res 41(6), 795–802.
- Petroff OAC, Pritchard JW, Behar KL et al. (1985) Cerebral intracellular pH by 31P magnetic resonance spectroscopy. Neurology 35, 781–788.
- Podo F, Henriksen O and Bovee WM et al. (1998) Absolute metabolite quantification by in vivo NMR spectroscopy: I. Introduction, objectives and activities of a concerted action in biomedical research. Magn Reson Imaging 16(9), 1085–1092.
- Portman MA and Ning X (1990) Developmental adaptations in cytosolic phosphate content and pH regulation in the sheep heart in vivo. J Clin Invest 86, 1823–1828.
- Pritchard J, Rothman D, Novotny E et al. (1991) Lactate rise detected by 1H NMR in human visual cortex during physiologic stimulation. Proc Natl Acad Sci USA 88: 5829–5831.
- Pysh JJ (1970) Mitochondrial changes in rat inferior colliculus during postnatal development: an electron microscopic study. Brain Res 18, 325–342.
- Rai GS, McConnell JR, Waldman A et al. (1999) Brain proton spectroscopy in dementia: an aid to clinical diagnosis. Lancet 353(9158), 1063–1064.
- Robbins RC, Balahan RS and Swain JA (1990) Intermittent hypothermic asanguineous cerebral perfusion (cerebroplegia) protects the brain during prolonged circulatory arrest. A phosphorous 31 nuclear magnetic resonance study. J Thoracic Cardiovasc Surg 99, 878–884.
- Robertson NJ and Edwards AD (1998) Recent advances in developing neuroprotective strategies for perinatal asphyxia. Curr Opin Pediatr 10, 575–580.
- Robertson NJ, Cox IJ, Cowan FM et al. (1999) Cerebral intracellular lactic alkalosis persisting months after neonatal encephalopathy measured by magnetic resonance spectroscopy. Pediatr Res 46(3), 287–296.
- Robertson NJ, Cox IJ, Cowan FM et al. (1999) Brain intracellular pH measured by phosphorus-31 MRS correlates with outcome after perinatal asphyxia. [Abstract] Early Hum Dev 54(1), 76–77.
- Robertson NJ, Lewis RH, Cowan FM at al. (2001) Early increases in brain myo-inositol measured by proton magnetic resonance spectroscopy in term infants with neonatal encephalopathy. Pediatr Res (in press).
- Robertson NJ, Kuint J, Counsell TJ et al. (2000) Characterisation of cerebral white matter damage in preterm infants using 1H and 31P magnetic resonance spectroscopy. J Cereb Blood Flow Metab 20: 1446–1456.
- Robitaille PM, Abduljalil AM, Kangarlu A et al. (1998) Human magnetic resonance imaging at 8 T. NMR Biomed 11, 263–265.
- Ross BD (1991) Biochemical considerations in 1H spectroscopy. Glutamate and glutamine: myo-inositol and related metabolites. NMR in Biomedicine 4, 59–63.
- Roth SC, Edwards AD, Cady EB et al. (1992) Relation between cerebral oxidative metabolism following birth asphyxia and neurodevelopmental outcome and brain growth at one year.Dev Med Child Neurol 34, 285–295.
- Roth SC, Baudin J, Cady EB et al. (1997) Relation of deranged neonatal cerebral oxidative metabolism with neurodevelopmental outcome and head circumference at 4 years. Dev Med Child Neurol 39, 718–725.
- Shonk T and Ross BD (1995) Role of increased cerebral myo-inositol in the dementia of Down Syndrome. Mag Reson Med 33, 858–861.
- Sunagawa S, Buist RJ, Hruska FE et al. (1994) Hydrogen ion compartmentation during and following cerebral ischaemia evaluated by 31P NMR spectroscopy. Brain Res 641, 328–332.
- Thurston JH, Sherman WR, Hauhart RE et al. (1989) myo-Inositol: a newly identified nonnintrogenous osmoregulatory molecule in mammalian brain. Pediatr Res 26, 482–485.
- Toft PB, Christiansen P, Pryds O et al. (1994) T1, T2, and concentrations of brain metabolites in neonates and adolescents estimated with H-1 MR spectroscopy. J Magn Reson Imaging 4, 1–5.
- Toft PB, Leth H, Peitersen B et al. (1997) Metabolic changes in the striatum after germinal matrix haemorrhage in the preterm infant. Pediatr Res 41(3), 309–316.
- Urenjak J, Williams SR, Gadian DG et al. (1992) Specific expression of N-acetylaspartate in neurons, oligodendrocyte-type 2 astrocyte progenitors and immature oligodendrocytes in vitro. J Neurochem 59, 55–61.
- van der Knapp MS, van der Grond J, van Rijen PC et al. (1990) Age-dependent changes in localised proton and phosphorus MR Spectroscopy of the brain. Radiology 176, 509–513.
- Vicario C, Arizmendi C, Malloch G, et al. (1991) Lactate utilization by isolated cells from early neonatal rat brain. J Neurochem 57: 1700–1707.
- Videen JS, Michae IT, Pinto P et al. (1995) Human cerebral osmolytes during chronic hyponatraemia. A proton magnetic resonance spectroscopy study. J Clin Invest 95, 788–793.
- Volpe JJ (1998) Neurologic outcome of prematurity. Arch Neurol 55, 297–300.
- Volpe JJ and Volpe JJ (Eds) (1995) Neurology of the Newborn, 3rd edn. Philadelphia, Saunders, pp. 634–671.
- Yager JY, Brucklacher RM and Vannucci RC (1992) Cerebral energy metabolism during hypoxia–ischemia and early recovery in immature rats. Am J Physiol 262, H672–677.
- Yamashita T, Kohmura E, Yamauchi A et al. (1996) Induction of SMT mRNA after focal cerebral ischaemia: evidence for extensive osmotic stress in remote areas. J Cerebral Blood Flow Metab 16, 1203–1210.
- Yamashita T, Shimada S, Yamauchi A et al. (1997) Induction of SMIT mRNA after rat cryogenic injury. Mol Brain Res, 46, 236–242.
- Yamashita T, Yamauchi A, Miyai A et al. (1999) Neuroprotective role of SMIT against veratidine cytotoxicity. J Neurochem 72, 1864–1870.
- Younkin D, Medoff-Cooper B, Guillet R et al. (1988) In vivo 31P nuclear magnetic resonance measurement of chronic changes in cerebral metabolites following neonatal intraventricular haemorrhage. Pediatrics 82(3), 331–336.